Introduction
What is the preanalytical phase?
Laboratory testing has been described as a “Brain-to-Brain Loop”(1,2) that starts when the ordering provider conceives of ordering a test and ends when the ordering provider interprets and acts upon the results of the test. Additionally, the laboratory testing process has been traditionally divided into three phases. Testing begins in the preanalytical phase, which includes all activities that occur prior to the sample’s insertion into the analytical instrument. The analytical phase follows, and includes the chemical reactions, fluidics, and other processes that occur in the analytic platform. Testing finishes with the post-analytical phase, which includes all events occurring after the test result is generated, such as data entry, transport of the result through various information systems, and interpretation of the result.
It has been recognized for years that the phase of testing most prone to errors is the preanalytical phase (3-8). Plebani provides a very detailed outline of pre-analytical errors, a significant number of which are applicable to blood gas analysis (8). To provide context for the discussion here, the major errors discussed in that review are shown in Table 1. Inasmuch as preanalytical (and postanalytical) processes occur outside of the clinical laboratory itself, it is both ironic and challenging to laboratory staff that a significant fraction of so-called “laboratory errors” do not occur in the laboratory. The reasons why preanalytical processes are more error-prone than processes in later testing phases are varied, as they include both patient factors, such as interferences in blood samples, and iatrogenic factors, such as the fact that specimen collection is an almost entirely manual process. To the contrary, analytical and post-analytical processes in the modern clinical laboratory are often automated, and thus reliable computer-based safeguards can be implemented. Some of the more common preanalytical errors, such as incorrect test orders, incorrect sample handling/collection, and specimen mislabeling are currently difficult to control with computerized or robotic solutions, and the interventions currently used to prevent these problems (protocols, training, checklists, double-checking, etc…) are unlikely to be 100% effective.
Table 1. Errors in the phases of laboratory testing, from (8). Preanalytical steps focused on in this review are highlighted in bold.
What is blood gas testing?
The term “Blood gas testing” traditionally refers to determining the partial pressures of the physiologically active gases in blood (pO2, pCO2), the blood pH, and the oxygen saturation of hemoglobin (SaO2) (9). As analytical instrumentation has improved and additional simultaneous analyses have been included on analytical instruments, however, the scope of what is included under the guise of “blood gas testing” has expanded. The methods for and importance of blood gas testing for pO2 and pCO2 were first described by Van Slyke in the early 1900s (10), but current commercially-available instruments are capable of performing hemoglobin quantitation and cooximetry, as well as measuring electrolytes (sodium, potassium, chloride, ionized calcium and magnesium), glucose, lactate, and creatinine, usually simultaneously (11,12). Additionally, testing platforms have now been miniaturized sufficiently to allow point-of-care (POC) testing in emergency departments and surgical suites, as well as in the community by first responders (13). Thus, what is commonly referred to as a “blood gas” analysis may include a few or many tests, and may be performed in a central laboratory, at the point of care in a clinic, or even in a helicopter transporting an injured patient from an accident. To keep the scope of this review manageably narrow, preanalytical considerations of “blood gas” testing will be discussed only in regards to the simultaneous determination of blood pH, oxygen saturation, pO2, and pCO2 performed in a laboratory or at the point of care.
Preanalytical considerations in blood gas testing
The brain-to-brain loop as described by Lundberg (1) contains many preanalytical steps, each of which is prone to errors. A discussion of each sub-phase of the preanalytical process in blood gas testing (ordering, identification, collection, transportation, separation/preparation), along with the relevant considerations and potential pitfalls of each step, follows here. Note that many preanalytical issues, such as the importance of accurate specimen labeling, are not unique to blood gas testing.
Ordering
The decision whether or not to order a blood gas test is beyond the scope of a review such as this, but understanding the rational basis for blood gas test utilization in critically ill patients is helpful. Common indications for arterial blood gas testing have been reviewed elsewhere (14). The most common indications include critical illnesses with pathophysiologic changes that alter gas exchange or acid-base balance. While testing of ambulatory patients is sometimes indicated, the illnesses that require blood gas analysis are most often found in hospitalized patients being treated at the highest levels of intensive care. Just because the testing is common in critically ill patients, however, does not necessarily mean that it is needed in all critically ill patients. Test overutilization stemming from repetitive testing for many analytes at daily or more frequent intervals is an unfortunate hallmark of intensive care in resource-rich settings, even though there is scant evidence to support this frequency of testing for all analytes, and there is ample evidence that test utilization can be substantially reduced with no apparent adverse effects (15-23). As blood gas testing has alone been found to contribute significantly to iatrogenic anemia in critically ill patients, accounting for nearly 40% of the blood loss in one study (24,25), assuring that all tests ordered are absolutely necessary should be a primary concern.
In a critical care setting, blood gas testing is often utilized primarily for management of mechanically ventilated patients to assess oxygenation and ventilation. While some existing guidelines for managing specific disorders (i.e. chronic obstructive pulmonary disease (26)) indicate a suggested role for blood gas analysis, and some older general guidelines exist from the American Association for Respiratory Care (27), the major relevant professional societies do not offer recent guideline statements addressing the use of blood gas analysis in all situations. In the author’s institution, as an example of a common clinical practice, critical care physicians generally request an arterial blood gas (ABG) every morning for intubated patients, after ventilator settings changes that involve changes in minute ventilation (rate or tidal volume), and whenever the physicians judge it to be necessary based on other clinical parameters. While noninvasive pulse oximetry and capnometry are usually used for continuous monitoring, they are not considered to be replacements for ABG testing, which is preferred for definitive assessment of ventilation and oxygenation (28).
CLSI guidelines (C46-A2) (9) indicate several analytical justifications for repeating an analysis, including when a previous analysis is inconsistent with a previous result or condition, internally inconsistent (i.e. pO2 impossibly high for a patient breathing room air, or pH inconsistent with pCO2 and bicarbonate), or at the extremes of a range of expected values. It should be noted that there are no universally accepted guidelines for the frequency of blood gas testing in any specific clinical situation, that practices likely vary widely around the world, and that manufacturers of capnometers and pulse oximeters market their devices partly on the basis that their use can reduce the need for ABG testing. The ultimate decision to perform a blood gas test lies with the clinician, and thus a good working relationship between the laboratory director and clinical staff and ample educational resources are paramount in ensuring appropriate test utilization.
Identification
Perhaps more so than for other chemistry analysis, correct identification of the patient is extremely important for downstream interpretation because an immediate action is often warranted after blood gas results are returned. At the very least, a specimen collected for blood gas analysis should be positively identified with unique patient identifiers, the time of collection, and the patient location to ensure that the correct patient is undergoing testing, and that a critical result can be phoned for immediate action. As for any other specimen obtained for clinical laboratory analysis, the more that specimen identification and downstream labeling can be automated, such as through bar-coded wristbands/tubes and automated sorters and aliquotters, the better.
Additional required information includes the patient’s temperature (chemical equilibria shift at different temperatures), the site of sampling (arterial vs. venous, or anatomic site), FIO2 or description of oxygen delivery (i.e. 100% O2 by facemask), ventilator status (spontaneous vs. mechanical ventilation), and time since last ventilator change (which should be at least 30 minutes) (29). If the patient is undergoing dynamic testing, then the activity status of the patient (lying down, exercising) is also required. For definitive interpretation of results, it is also essential to know the clinical status of the patient at the exact moment of specimen collection. Even seemingly minor issues such as anxiety over specimen collection can alter results by increasing the respiration rate (29).
Collection
Because the biologic variability of some blood gas parameters are quite low (pH, pC02), very little error resulting from specimen collection can be tolerated if one is to be able to interpret small, but real, changes in analyte concentrations (30). Additionally, because blood gas testing must be performed on whole blood, and because the results of testing are often required urgently, little specimen manipulation is required or desired, and specimens are most often collected expressly for the single purpose of blood gas testing.
Blood gas testing can be performed either on arterial or venous (VBG) blood, and the choice of sample type depends on the clinical question asked. A VBG can be obtained from peripheral veins, central veins, or pulmonary artery in a catheterized patient, and certain analytes when measured in central venous blood (pH and pCO2) correlate well with their arterial counterparts, with small but reproducible biases (31). Mixed venous or central venous blood gas analysis, for example, is recommended to be performed in treatment of sepsis, as targeting an oxygen saturation (ScvO2) above 70% in these patients has a proven mortality benefit (32,33). While older guidelines from the IFCC (29) indicate that venous blood gas samples should only be used for analysis of electrolytes, bicarbonate, base excess, hemoglobin, and hematocrit, the newer data cited here (31,33) makes it clear that VBG assessment of oxygen saturation, pCO2, and pH can be a useful clinical tool.
While the gold standard sample for blood gas testing is generally considered to be arterial blood collected anaerobically from an indwelling arterial catheter (usually radial artery from an adult, or perhaps umbilical in a neonate) or arterial puncture, arterial sample is somewhat more difficult, painful, and dangerous than routine venipuncture (9). Therefore, there has been interest in a less painful and risky sampling procedure, and because venous blood is not a satisfactory substitute for arterial blood for routine blood gas testing (9), capillary blood sampling has been investigated. Capillary blood gas analysis is particularly desirable in neonates, for whom vascular access can be challenging and for whom blood collection with standard approaches can withdraw volumes of blood that are unacceptably large relative to the total blood volume. Capillary blood gas analysis has been reviewed previously (34), a CLSI document regarding the practice of capillary blood collection exists (H04-A6) (35), and somewhat older IFCC recommendations cover this practice as well (29). In this procedure, the capillary bed of a skin site, usually the finger, heel or earlobe, is dilated with heat or a topical vasodilator to “arterialize” the blood in the area. Next, the skin is punctured, and then a sample of capillary blood (which is actually an ill-defined mixture of capillary blood, arteriolar blood, venular blood, interstitial fluid, and intracellular fluid) is collected from the center of the resulting blood drop with a collection device, which is most often a heparinized capillary glass tube (9). Major controversies in this area include the degree of correlation of between arterial and capillary results and which “arterialization” technique, if any, to employ prior to blood collection. There is consensus that pH and pCO2correlate fairly well between arterial and capillary samples because of the low arteriovenous gradient of these analytes, but pO2 correlates significantly less well.
The sample collection container is a special consideration in blood gas testing. Most importantly, gas-tight syringes are required, rather than evacuated tubes, since oxygen and carbon dioxide cannot be allowed to enter or leave the sample. Glass syringes were the predominant collection devices in the past, but most health care facilities now use plastic syringes due to safety concerns with glass breakage. The plastics used for blood gas collection syringes are partially gas permeable, and this permeability can increase at lower temperature (36-39). The reason for this effect has been hypothesized to be a low temperature induced contraction of the polymer molecules in plastic tubes, leading to a widening in the atomic-scales pores in the material; these pores would need to be large enough to allow oxygen to escape, but too small for the larger carbon dioxide molecules to transit, since pCO2 does not change significantly in samples in chilled plastic tubes. The practice of keeping glass collection syringes on ice after collection, which was historically performed to prevent metabolic consumption of oxygen (see below), is thus no longer recommended for plastic syringes. This gas permeability issue will bring the pO2 in a sample closer to the ambient value of ~150 mmHg (20 kPa), which may be a rise or a fall depending on the starting pO2 value. A related problem is the issue of air aspiration or bubble formation in blood gas syringes. Even small bubbles, after equilibration with a blood sample, can significantly alter blood gas analyte concentrations. The typical findings in a blood gas sample exposed to air would be a spurious elevation or drop in pO2 to ~150 mm Hg (20 kPa), and perhaps a decrease in pCO2 and an increase in pH due to loss of carbonic acid if the exposure to air is prolonged. Increased pH can further lead to other analytical errors seen in expanded blood gas panels, such as decreased free/ionized calcium because of increasing calcium-protein binding (40).
One of the more common sources of interference in laboratory testing, hemolysis, is introduced during collection by shearing of red cells, and it is a potential source of interference in blood gas testing (41,42). If potassium concentrations are sought in addition to blood gas parameters, then the release of highly concentrated potassium from lysed red blood cells can contribute to a positive error, and if hemolysis is massive, then analytes less concentrated inside cells (sodium, calcium) may be diluted. The values of gas partial pressures and pH, however, are not greatly affected by hemolysis because large intracellular-extracellular gradients are not present. Massive hemolysis can contribute to an error in hematocrit for a device that measures hematocrit by conductance, but would not be expected to introduce an error in instruments that calculate hematocrit from the measured hemoglobin concentration, since the hemoglobin concentration is usually determined after the specimen is hemolyzed by the instrument.
The choice of anticoagulant for blood gas analysis can affect the measured results. Usually, blood gas syringes are preloaded with a measured amount of lyophilized heparin that has been pre-titrated with cations (i.e. “balanced”). Balanced heparin takes into account the fact that heparin is a polyanion that can bind cation electrolytes, so physiologic concentrations of electrolytes such as calcium are added to minimize errors due to cation exchange with heparin. Liquid heparin solutions are not preferred because short sample collections can lead to an improper mix of anticoagulant and blood (43). If too much liquid heparin is present, it could dilute out some analytes such as bicarbonate and pCO2 (29,44), or affect pO2 results because the liquid heparin itself has atmospheric pO2 (150 mmHg/20 kPa).
Anticoagulants other than heparin (EDTA, oxalate) are not commonly used in blood gas testing, as they can interfere with electrolyte or enzymatic measurements by chelating divalent cations. A large list of potential interfering substances in sample collection tubes and devices is available in Bowen et al. (45), with the primary interferences of relevance to blood gas testing including oxygen-permeability of tubes, hemolysis due to alcohol disinfectants, small bore needles, narrow catheters, and excessive syringe suction, and interferences due to inappropriate anticoagulants. Additionally, contamination of a sample drawn from an indwelling catheter with resident fluid in the line, a so-called “line draw”, is an important source of dilutional error in many types of laboratory testing including blood gas testing.
Transportation
As mentioned briefly above, when a whole blood sample is transported, living cells in the sample are still in contact with the nutrients (glucose, oxygen) in the plasma, and metabolism can proceed freely for some time. Over a period, though, a significant quantity of the oxygen and glucose will be consumed, and as the sample becomes anaerobic, lactate will be produced with a concomitant acidosis. Obviously, for an accurate assessment of the blood gas parameters occurring in the patient, and not just in the sample, these metabolic changes must be minimized. Because storage on ice is contraindicated for the reasons mentioned above, one obvious step that can be taken to counter this potential source of error is simply to analyze specimens quickly. The IFCC thus recommends keeping transportation time minimal, and analyzing samples collected in plastic syringes and transported at room temperature within 15 minutes if pO2 or oxygen saturation are desired, and 30 minutes otherwise (29).
CLSI guidelines (C46-A2) (9) further describe several factors to consider regarding transport of blood gas specimens. Transfer by hand is generally acceptable, and exposure to air is obviously discouraged. One key transportation factor that is mostly relevant to central laboratory blood gas testing, but not to POC testing, is the issue of specimen transport by a pneumatic tube system. One study has shown significantly higher pO2 results on ABG testing when a sample has been transported by pneumatic tube vs. manual transport (46). One reason for this finding is that during this mode of transport, which is commonly used between distant hospital sites and central laboratories, blood samples can be vigorously shaken and exposed to up to 15 × g acceleration (47). In one study, most blood gas parameters were unaffected by these large forces, but significant increases in indicators of hemolysis (potassium, AST, LD) were noted (47). Of interest in this study, though, was that the most significant parameter affecting test results out of the many investigated was sample acceleration. Other studies have demonstrated clinically significant alterations in pO2 after pneumatic tube transport, likely due to accelerated equilibration of small air bubbles with the sample during vigorous shaking (48-50).
Separation/preparation
Blood gas analysis, as commonly performed, does not require specimen separation by centrifugation. Conversely, it is performed on whole blood, so complete mixing after collection is required to ensure adequate anticoagulant dispersal in the sample, and additional mixing prior to analysis is required to reverse any erythrocyte settling that may have occurred prior to analysis.
Physiologic and iatrogenic considerations in blood gas testing
As with any laboratory test, the conditions of patients themselves can affect the results of laboratory tests in a way that could be misleading to whoever must interpret the test. Table 2 lists several possible physiologic and iatrogenic interferences that may be common in hospitalized patients.
Table 2. Physiologic or iatrogenic confounders in blood gas measurement.
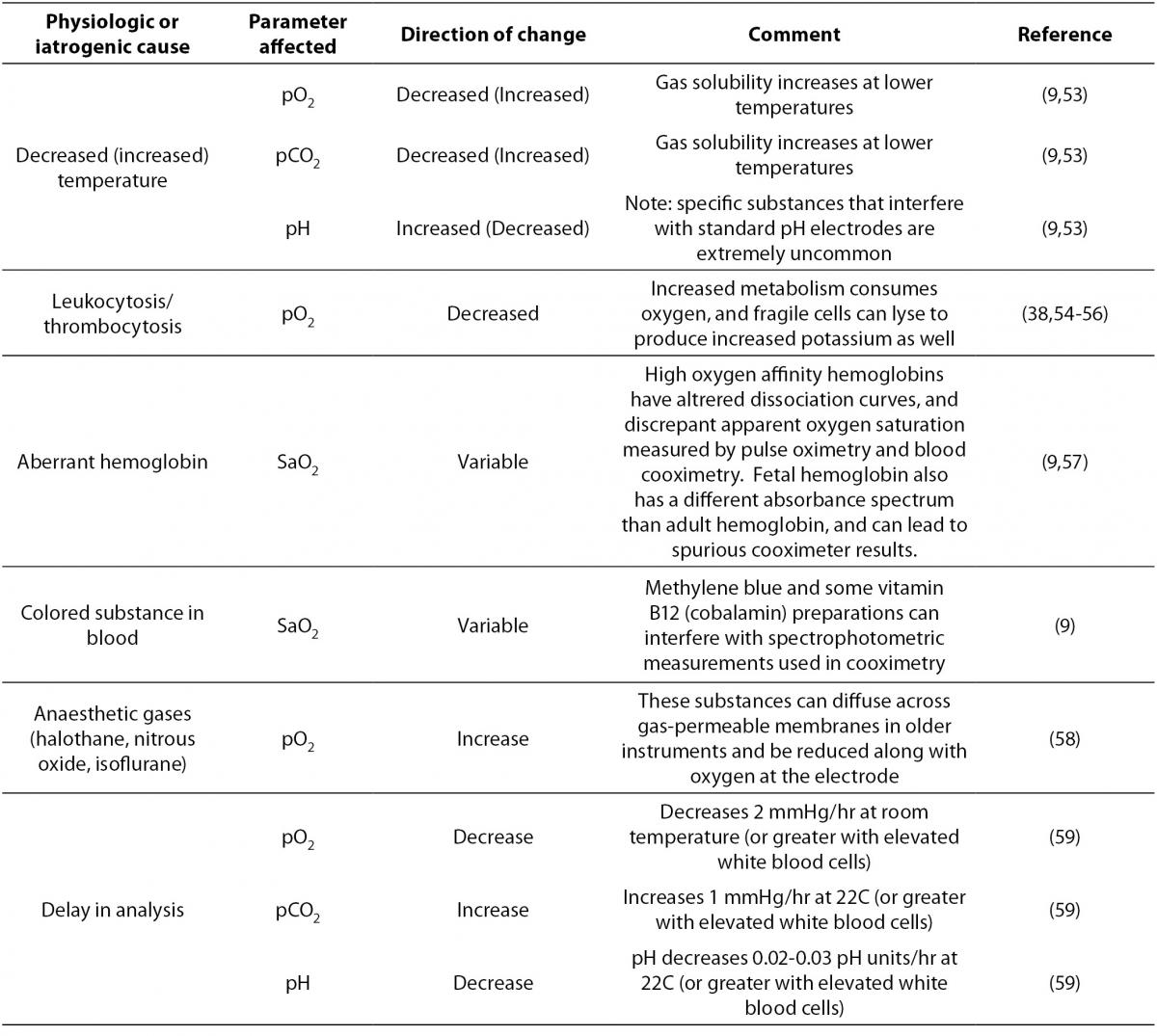
How one should deal with temperature correction of blood gas results is challenging, because while pO2, pCO2 and pH all change with temperature, instruments generally measure these analytes at 37 °C. Thus, patients with body temperatures at or near 37 °C will have accurate results that correspond to the reference ranges (which were defined using normothermic volunteers), whereas febrile or extremely hypothermic patients may have results that differ quite markedly from the true in vivo concentrations of the analytes in question, and the relevance of normothermic reference ranges is unclear. Unfortunately, whether or not to use temperature-corrected values at all, and if so, how to apply the results of temperature correction clinically, are both controversial topics and beyond the scope of this review (9). As an example of how a clinical laboratory could confront this controversy, in the author’s institution, both the values measured at 37 °C as well as the values calculated to be present at the patient’s indicated temperature are reported, and it is left up to the clinician to decide which value to use. In general, when a temperature corrected value is reported, the uncorrected value should also be reported.
POC Considerations
Testing at the point of care has several advantages over central laboratory testing, namely decreased total turnaround time and a lower (but still non-zero) likelihood of specimen misidentification. Because the very nature of blood gas testing implies that an immediate or urgent response to the results is required, the advantages in turnaround time make POC testing quite common in operating rooms, emergency rooms, and in the field (i.e. as part of a rescue or emergency response). One consideration for POC testing in the field that is not immediately obvious to those employed in a central laboratory is that first responders may not measure blood gases in the same ambient conditions that are present in the central laboratory. In the author’s institution, for example, the affiliated air rescue organization performs extensive POC blood gas testing in mountainous regions and in the air during medical evacuation flights, and the ambient pressure at elevated altitudes, as well as the partial pressure of oxygen in air, can be 25% lower than at sea level (51,52). Thus, when interpreting the results of POC tests, one must remember to consider where the “point of care” actually is, and to correct one’s expectations for normal values accordingly.
Summary
Control of preanalytical variables in blood gas testing is critical for ensuring an accurate and prompt response to what is often an urgent test. While the common preanalytical errors, such as specimen mislabeling and incorrect sampling, can affect blood gas tests in the same way as other laboratory tests, blood gas testing is special in that some analytes are gaseous and thus vulnerable to an expanded set of errors and interferences. Understanding these effects, including those due to physiology, pathophysiology, and extrinsic human factors, is essential for providing actionable information to the clinicians who take care of our patients. In the author’s opinion, the most important factors to consider in the preanalytical process are ensuring accurate patient identification, performing correct sampling techniques, using recommended sample containers and anticoagulants, and allowing minimal time between sampling and analysis.
Notes
Potential conflict of interest
None declared.
References
7. Astion ML, Shojania KG, Hamill TR, Kim S, Ng VL. Classifying laboratory incident reports to identify problems that jeopardize patient safety. Am J Clin Pathol 2003;120:18-26.
http://dx.doi.org/10.1309/8U5D0MA6MFH2FG19.
9. CLSI. Blood gas and ph analysis and related measurements; approved guideline - second edition. Vol. CLSI Document C46-A2. Wayne, PA: Clinical and Laboratory Standards Institute, 2010.
11. Skurup A, Kristensen T, Wennecke G, Group NKDEPLW. New creatinine sensor for point-of-care testing of creatinine meets the national kidney disease education program guidelines. Clin Chem Lab Med 2008;46:3-8.
http://dx.doi.org/10.1515/CCLM.2008.004.
12. Bénéteau-Burnat B, Pernet P, Pilon A, Latour D, Goujon S, Feuillu A, Vaubourdolle M. Evaluation of the gem premier 4000: A compact blood gas co-oximeter and electrolyte analyzer for point-of-care and laboratory testing. Clin Chem Lab Med 2008;46:271-9.
http://dx.doi.org/10.1515/CCLM.2008.043.
13. De Koninck AS, De Decker K, Van Bocxlaer J, Meeus P, Van Hoovels L. Analytical performance evaluation of four cartridge-type blood gas analyzers. Clin Chem Lab Med 2012;50:1083-91.
14. Raffin TA. Indications for arterial blood gas analysis. Ann Intern Med 1986;105:390-8.
15. May TA, Clancy M, Critchfield J, Ebeling F, Enriquez A, Gallagher C, et al. Reducing unnecessary inpatient laboratory testing in a teaching hospital. Am J Clin Pathol 2006;126:200-6.
http://dx.doi.org/10.1309/WP59YM73L6CEGX2F.
16. Studnicki J, Bradham DD, Marshburn J, Foulis PR, Straumfjord JV. A feedback system for reducing excessive laboratory tests. Arch Pathol Lab Med 1993;117:35-9.
17. Calderon-Margalit R, Mor-Yosef S, Mayer M, Adler B, Shapira SC. An administrative intervention to improve the utilization of laboratory tests within a university hospital. Int J Qual Health Care 2005;17:243-8.
http://dx.doi.org/10.1093/intqhc/mzi025.
18. Attali M, Barel Y, Somin M, Beilinson N, Shankman M, Ackerman A, Malnick SD. A cost-effective method for reducing the volume of laboratory tests in a university-associated teaching hospital. Mt Sinai J Med 2006;73:787-94.
20. Wang TJ, Mort EA, Nordberg P, Chang Y, Cadigan ME, Mylott L, et al. A utilization management intervention to reduce unnecessary testing in the coronary care unit. Arch Intern Med 2002;162:1885-90.
http://dx.doi.org/10.1001/archinte.162.16.1885.
22. Cebul RD, Beck JR. Biochemical profiles. Applications in ambulatory screening and preadmission testing of adults. Ann Intern Med 1987;106:403-13.
26. (GOLD) GIfCOLD. From the global strategy for the diagnosis, management and prevention of copd. Vol.: Available from:
http://www.goldcopd.org/, 2011.
27. Aarc clinical practice guideline. Sampling for arterial blood gas analysis. American association for respiratory care. Respir Care 1992;37:913-7.
29. Burnett RW, Covington AK, Fogh-Andersen N, Külpmann WR, Maas AH, Müller-Plathe O, et al. International federation of clinical chemistry (ifcc). Scientific division. Committee on ph, blood gases and electrolytes. Approved ifcc recommendations on whole blood sampling, transport and storage for simultaneous determination of ph, blood gases and electrolytes. Eur J Clin Chem Clin Biochem 1995;33:247-53.
30. Harding PJ, Fraser CG. Biological variation of blood acid-base status: Consequences for analytical goal-setting and interpretation of results. Clin Chem 1987;33:1416-8.
31. Walkey AJ, Farber HW, O’Donnell C, Cabral H, Eagan JS, Philippides GJ. The accuracy of the central venous blood gas for acid-base monitoring. J Intensive Care Med 2010;25:104-10.
http://dx.doi.org/10.1177/0885066609356164
32. Dellinger RP, Levy MM, Carlet JM, Bion J, Parker MM, Jaeschke R, et al. Surviving sepsis campaign: International guidelines for management of severe sepsis and septic shock: 2008. Crit Care Med 2008;36:296-327.
http://dx.doi.org/10.1097/01.CCM.0000298158.12101.41
33. Rivers E, Nguyen B, Havstad S, Ressler J, Muzzin A, Knoblich B, et al. Early goal-directed therapy in the treatment of severe sepsis and septic shock. N Engl J Med 2001;345:1368-77.
http://dx.doi.org/10.1056/NEJMoa010307,
34. Higgins C. Capillary blood gases: To arterialize or not. MLO Med Lab Obs 2008;40:42, 4-7.
35. CLSI. Procedures and devices for the collection of diagnostic capillary blood specimens; approved standard - 6th edition. Vol. CLSI Document H04-A6. Wayne, PA: Clinical Laboratory Standards Institute, 2008.
36. Smeenk FW, Janssen JD, Arends BJ, Harff GA, van den Bosch JA, Schönberger JP, Postmus PE. Effects of four different methods of sampling arterial blood and storage time on gas tensions and shunt calculation in the 100% oxygen test. Eur Respir J 1997;10:910-3.
37. Beaulieu M, Lapointe Y, Vinet B. Stability of po2, pco2, and ph in fresh blood samples stored in a plastic syringe with low heparin in relation to various blood-gas and hematological parameters. Clin Biochem 1999;32:101-7.
http://dx.doi.org/10.1016/S0009-9120(98)00098-8.
38. Schmidt C, Müller-Plathe O. Stability of po2, pco2 and ph in heparinized whole blood samples: Influence of storage temperature with regard to leukocyte count and syringe material. Eur J Clin Chem Clin Biochem 1992;30:767-73.
39. Knowles TP, Mullin RA, Hunter JA, Douce FH. Effects of syringe material, sample storage time, and temperature on blood gases and oxygen saturation in arterialized human blood samples. Respir Care 2006;51:732-6.
43. Hedberg P, Majava A, Kiviluoma K, Ohtonen P. Potential preanalytical errors in whole-blood analysis: Effect of syringe sample volume on blood gas, electrolyte and lactate values. Scand J Clin Lab Invest 2009;69:585-91.
http://dx.doi.org/10.1080/00365510902878716.
44. Küme T, Sişman AR, Solak A, Tuğlu B, Cinkooğlu B, Coker C. The effects of different syringe volume, needle size and sample volume on blood gas analysis in syringes washed with heparin. Biochem Med (Zagreb) 2012;22:189-201.
46. Victor Peter J, Patole S, Fleming JJ, Selvakumar R, Graham PL. Agreement between paired blood gas values in samples transported either by a pneumatic system or by human courier. Clin Chem Lab Med 2011;49:1303-9.
http://dx.doi.org/10.1515/cclm.2011.611.
47. Streichert T, Otto B, Schnabel C, Nordholt G, Haddad M, Maric M, et al. Determination of hemolysis thresholds by the use of data loggers in pneumatic tube systems. Clin Chem 2011;57:1390-7.
http://dx.doi.org/10.1373/clinchem.2011.167932.
48. Astles JR, Lubarsky D, Loun B, Sedor FA, Toffaletti JG. Pneumatic transport exacerbates interference of room air contamination in blood gas samples. Arch Pathol Lab Med 1996;120:642-7.
49. Collinson PO, John CM, Gaze DC, Ferrigan LF, Cramp DG. Changes in blood gas samples produced by a pneumatic tube system. J Clin Pathol 2002;55:105-7.
http://dx.doi.org/10.1136/jcp.55.2.105.
50. Lu JY, Kao JT, Chien TI, Lee TF, Tsai KS. Effects of air bubbles and tube transportation on blood oxygen tension in arterial blood gas analysis. J Formos Med Assoc 2003;102:246-9.
51. Crapo RO, Jensen RL, Hegewald M, Tashkin DP. Arterial blood gas reference values for sea level and an altitude of 1,400 meters. Am J Respir Crit Care Med 1999;160:1525-31.
52. Bauer K. Prehospital airway management: High tech meets trauma: An air medical perspective. Crit Care Nurs Q 2012;35:281-91.
53. Ashwood ER, Kost G, Kenny M. Temperature correction of blood-gas and ph measurements. Clin Chem 1983;29:1877-85.
54. Khoo SM, Lee KH, Notley M. Spurious hypoxaemia in a patient with leukaemia and extreme leucocytosis. Singapore Med J 2003;44:208-10.
57. Verhovsek M, Henderson MP, Cox G, Luo HY, Steinberg MH, Chui DH. Unexpectedly low pulse oximetry measurements associated with variant hemoglobins: A systematic review. Am J Hematol 2010;85:882-5.
http://dx.doi.org/10.1002/ajh.21810.
58. Eberhard P, Mindt W. Interference of anesthetic gases at oxygen sensors. Birth Defects Orig Artic Ser 1979;15:65-74.
59. Scott M, LeGrys V, Klutts J. Eletrolytes and blood gases. In: Burtis C, Ashwood E, Bruns D, eds. Tietz textbook of clinical chemistry and molecular diagnostics, Vol. St. Louis, MO: Elsevier Saunders, 2006:1008.